What is an attosecond and what is it good for?
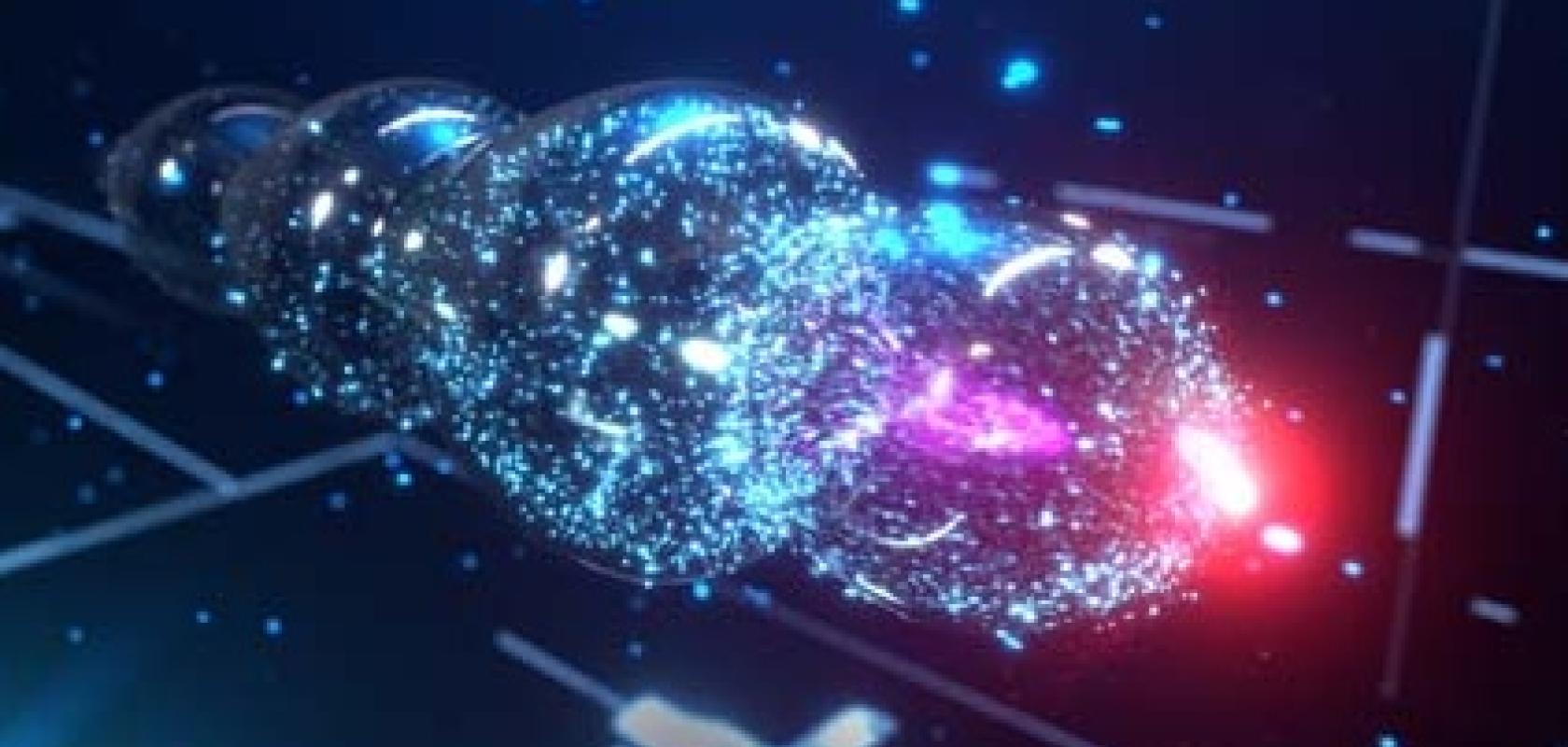
Attosecond pulses have already provided glimpses of fundamental ultrafast processes. Benjamin Skuse asks: what might be achieved if access were broadened to the wider world?
Register for FREE to keep reading
Join 15,000+ photonics professionals staying ahead with:
- Exclusive insights, funding alerts & market trends
- Curated newsletters and digital editions
- Access to The Photonics100 list of R&D champions
- Exclusive panels & roundtables for professional development
- Technical White Papers & product updates to guide smarter decisions
Sign up now
Already a member? Log in here
Your data is protected under our privacy policy.
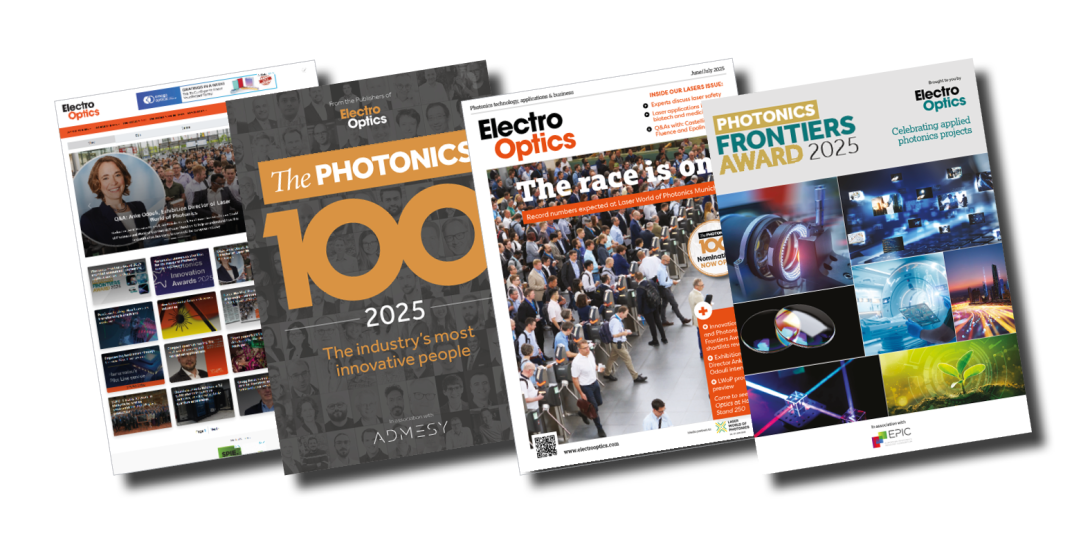